A story of a star - Part IV
Where we follow the protostar while it becomes a proper star, while encountering stellar birthplaces, different groups of stars and discover obscure denominations
Last time we talked about the depletion of deuterium, and now the status of the protostar is at stake. This happens because this object is not controlled anymore in its evolution by the free-fall timescale; instead, the timescale is now governed by the balance between gravity (which is always present) and the thermal properties of the star’s core. This new timescale goes by the name of Kelvin-Helmoltz timescale.
This timescale is way longer than the free-fall one: for instance, a molecular cloud of one solar mass takes 150 thousand years to arrive at the state of protostar that burns deuterium but takes 40 million years to contract into a proper star.
The road that protostars have to follow to reach the main-sequence is known as the Hayashi track, after C. Hayashi who demonstrated in 1961 that there is a boundary (a vertical line in the H-R diagram) that separates a stellar model that is indeed observed and those who are not observed (due to hydrostatic “impossibilities”).
This impossibility is because, at the right of the Hayashi track, no mechanism can transport the luminosity in the outer parts of the stellar envelope; on the other part of the track, convection, and radiation can indeed transport said luminosity.
The contraction time for protostars is heavily influenced by their initial masses. For a protostar of 0.8 solar masses, the time on the Hayashi track is something around 70 million years, whereas a protostar of 60 solar masses stays on the track for as short as 30 thousand years. The figure below shows the evolutionary tracks for a series of (simulated) stars: on the right is visible the vertical Hayashi track, which ends where luminosity reaches its minimum value. At this point radiative core of the star allows energy to travel into the (convective) envelope, and luminosity again increases. But the star is also shrinking, and then the temperature rises as well. At a certain point, the high temperature leads to the deuterium burning phase (the squares on the line). The long-dashed line represents the point where convection stops and the envelope of the star becomes radiative; whereas the short-dashed line represents the start of convection in the core.
After passing the deuterium-burning phase, the star has two main roads to produce energy via nuclear fusion: the PP chain and the CNO reactions: the former is the conversion of hydrogen into helium, whereas the latter converts carbon into nitrogen. Both reactions can happen in stars at the same time, but only one will dominate the other, ultimately setting the fate of the star.
In the phase on the plot where luminosity and temperature are rising, the core is at a temperature at which the CNO reactions are heavily favored, and thus these reactions dominate: the energy originated in such a way leads the core to expand. This leads to a decrease in total luminosity and also in the temperature (in the graph, this is noticeable near the short-dashed lines).
When carbon is exhausted in the core, the dominating reaction becomes the PP chain, which stabilizes the system and becomes the dominant source of energy for the star, which can now forget about gravitational energy while this source of energy, associated with the shrinking and the expanding of the star, becomes negligible (for now!).
Digression: little stars and where to find them
Not every star does reach the main-sequence phase.
If the mass of the protostar is less than 0.072 solar masses (72 times the mass of Jupiter) the core cannot reach the necessary temperature to ignite the nuclear reactions to stabilize the star.
Below this mass, some nuclear reactions still occur, nonetheless: at about 0.060 solar masses, the core can burn lithium1, whereas at 0.013 solar masses, it can burn deuterium.
If your favorite star has a mass ranging between 0.013 and 0.072 solar masses, astronomers classify it as a brown dwarf. Here’s one:
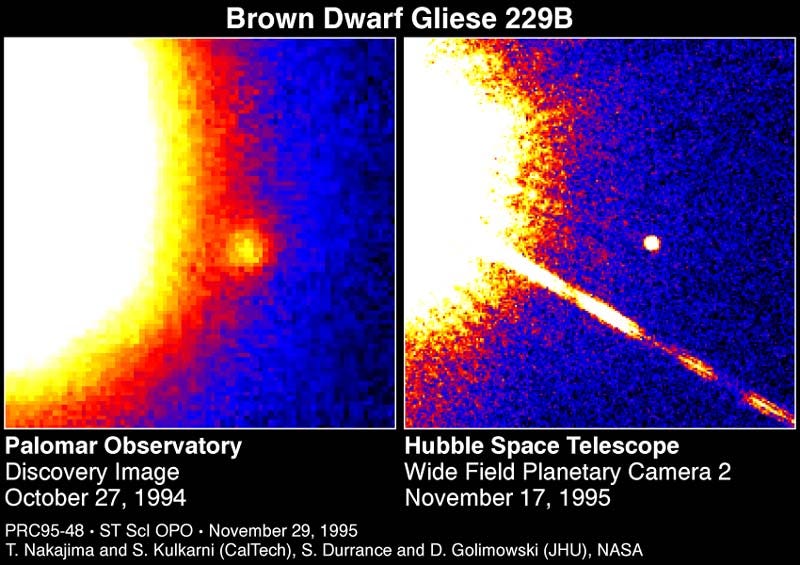
The image shows the very first brown star ever observed (in 1995); nowadays, hundreds of such objects have been observed, despite their low luminosity and their low radii, prevalently in the near-infrared part of the electromagnetic spectrum (and primarily in the Milky Way).
Stars at the Olympics: the start line of the main-sequence
As you can see from the plot at the start of this episode, each final point of every star track can be connected to the others, making them all reside on a diagonal line: this is called the zero-age main sequence (ZAMS), and it is the start of the hydrogen-burning phase of the star that renders it energetically stable.
This will be our starting point to follow the star in its evolution. But first, we have to talk about stellar groups.
Stellar consortia and space unions
H II regions
Massive stars heat their surroundings: they do so by emitting radiation primarily in the ultraviolet band of the electromagnetic spectrum. This radiation messes up with atoms in the cloud of gas surrounding the star, which afterward recombines with electrons to form neutral hydrogen; when doing so, more photons are created, this time in the visible band of the spectrum. These regions are called HII regions, and below there are some images obtained by different space telescopes.
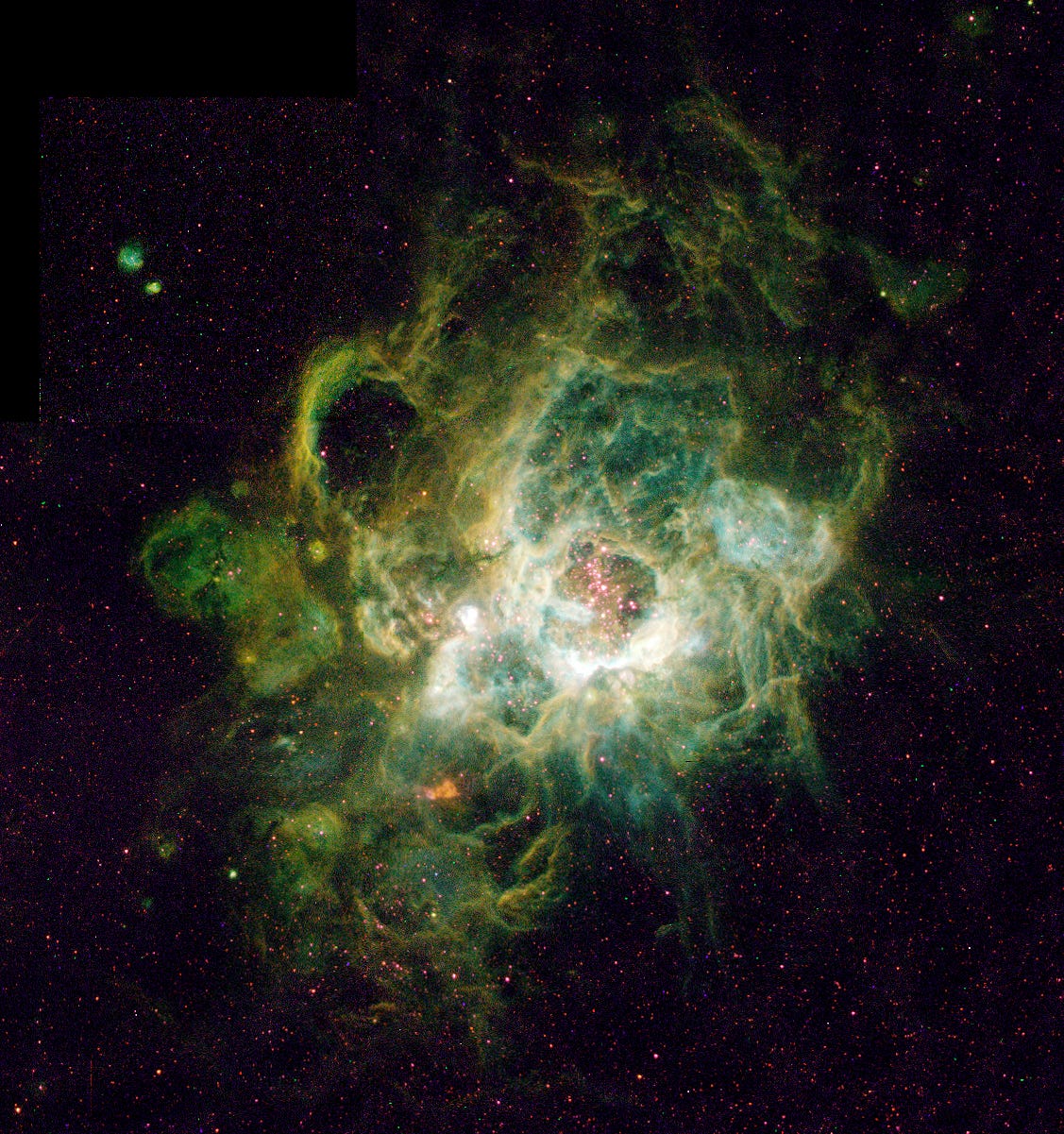
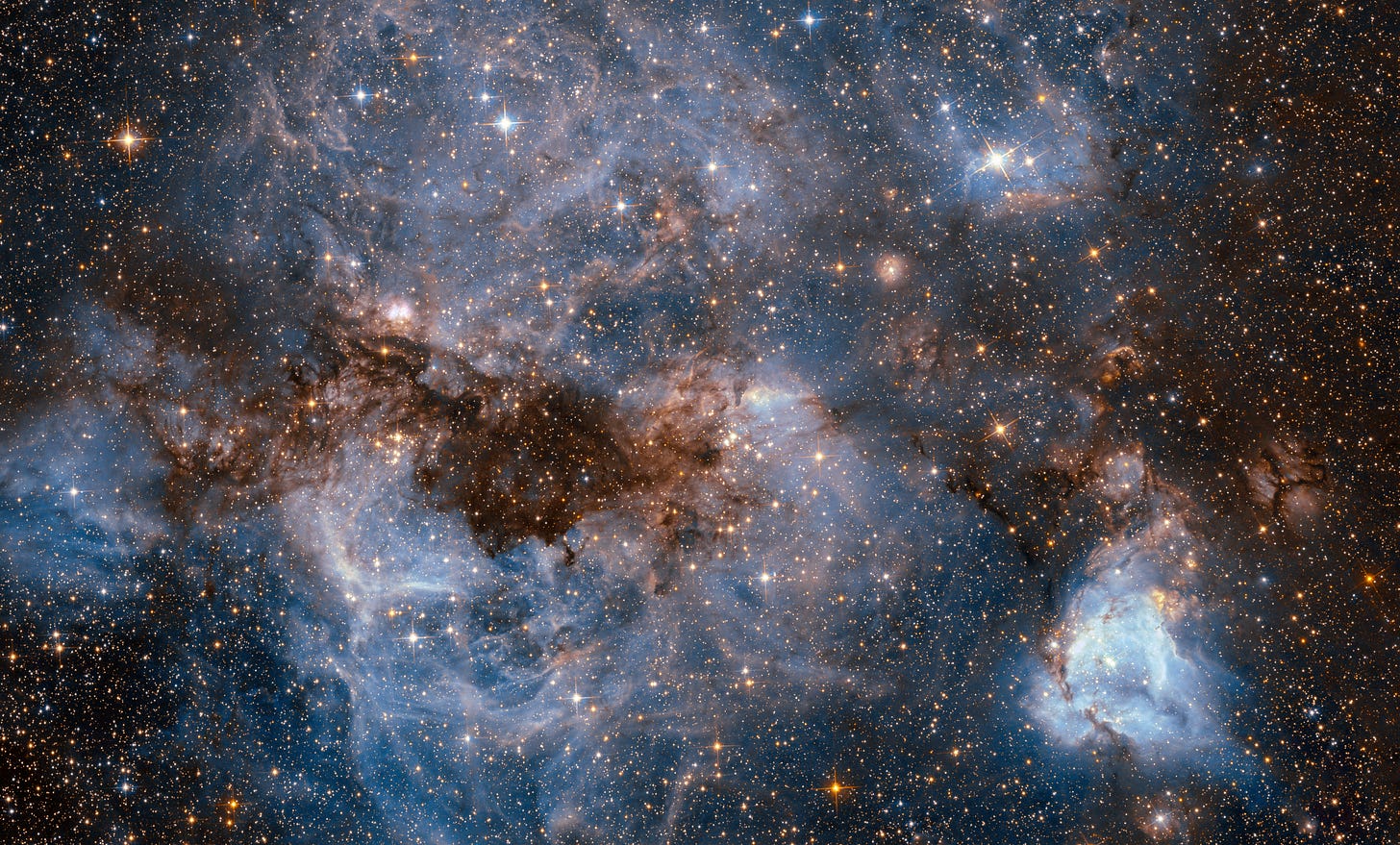
Sometimes it can happen that more massive stars, which drive radiation out in the cloud when forming, deplete the region of mass, necessary to form less massive stars. This is what happens for example at the Pillars of Creation, a giant structure in the Eagle Nebula (M16).
OB associations
These groups of stars are made primarily of O and B stars, and resemble a single structure, but are not gravitationally bound together: the velocities of stars is too high, and soon (in astronomical times, that is) these clusters of stars will become unbound.
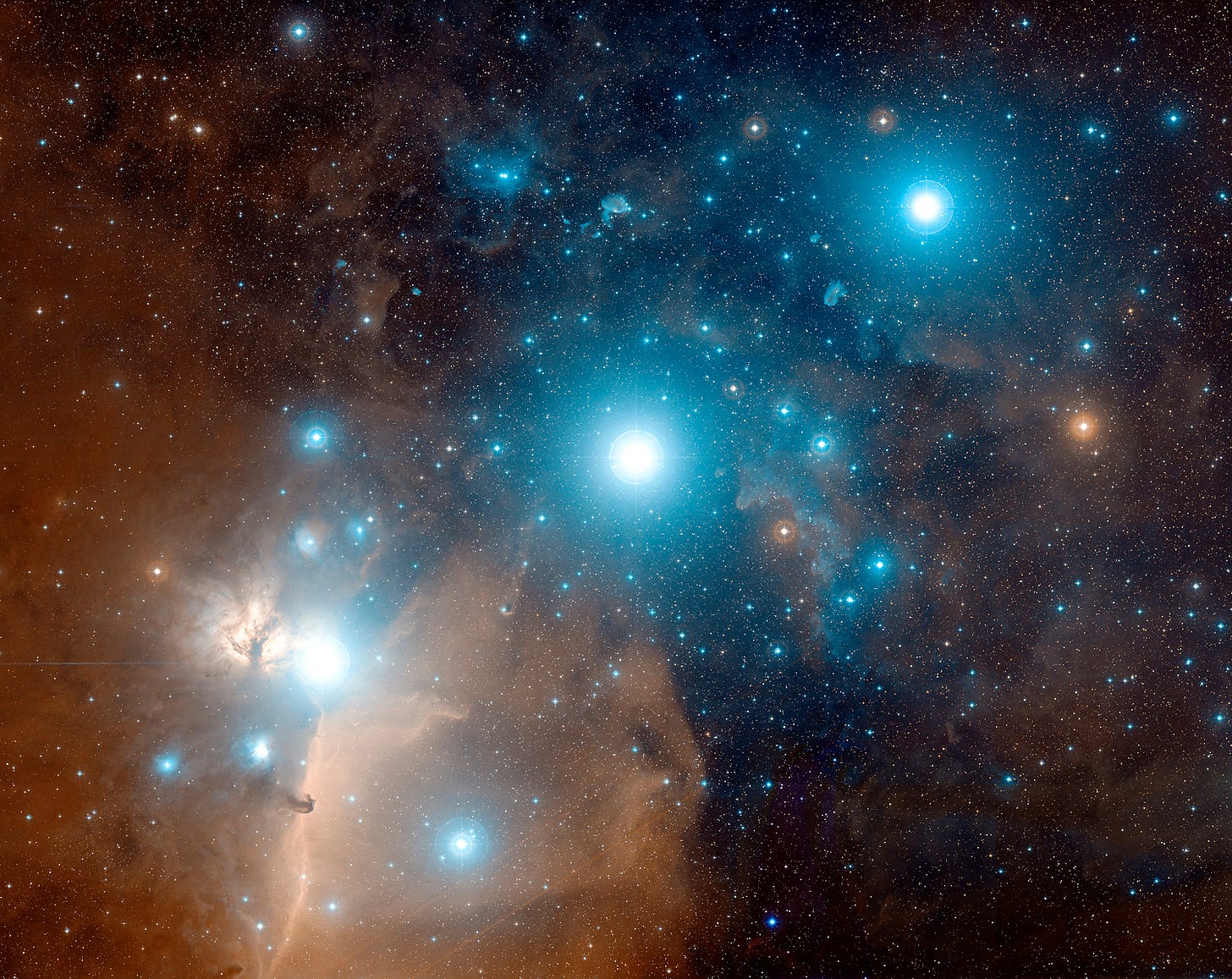
T Tauri stars
These kinds of stars show a high degree of variability in luminosity, with timescales of the order of days. They represent a class of pre-main-sequence stars and have masses in the range of 0.5 - 2 solar masses. One interesting property is the fact that they appear to be losing mass at a rate that is much more rapid (again, in astronomical timescales) than that of the Sun, for example.
They are called T Tauri because the prototype of this type of object is T Tauri, a star in the constellation Taurus (the one below):
FU Orionis stars
When a T Tauri star does not observe mass loss, but rather mass accretion, its luminosity increases This object is known as FU Orionis.
What will happen now?
In the next episode, we will encounter stars during their main sequence.
See you next time!
See you next time! (and if you liked subscribe!)
(hydrogen, helium, lithium, …)